Metal Hydride Hydrogen Storage Canisters: How Do They Work?
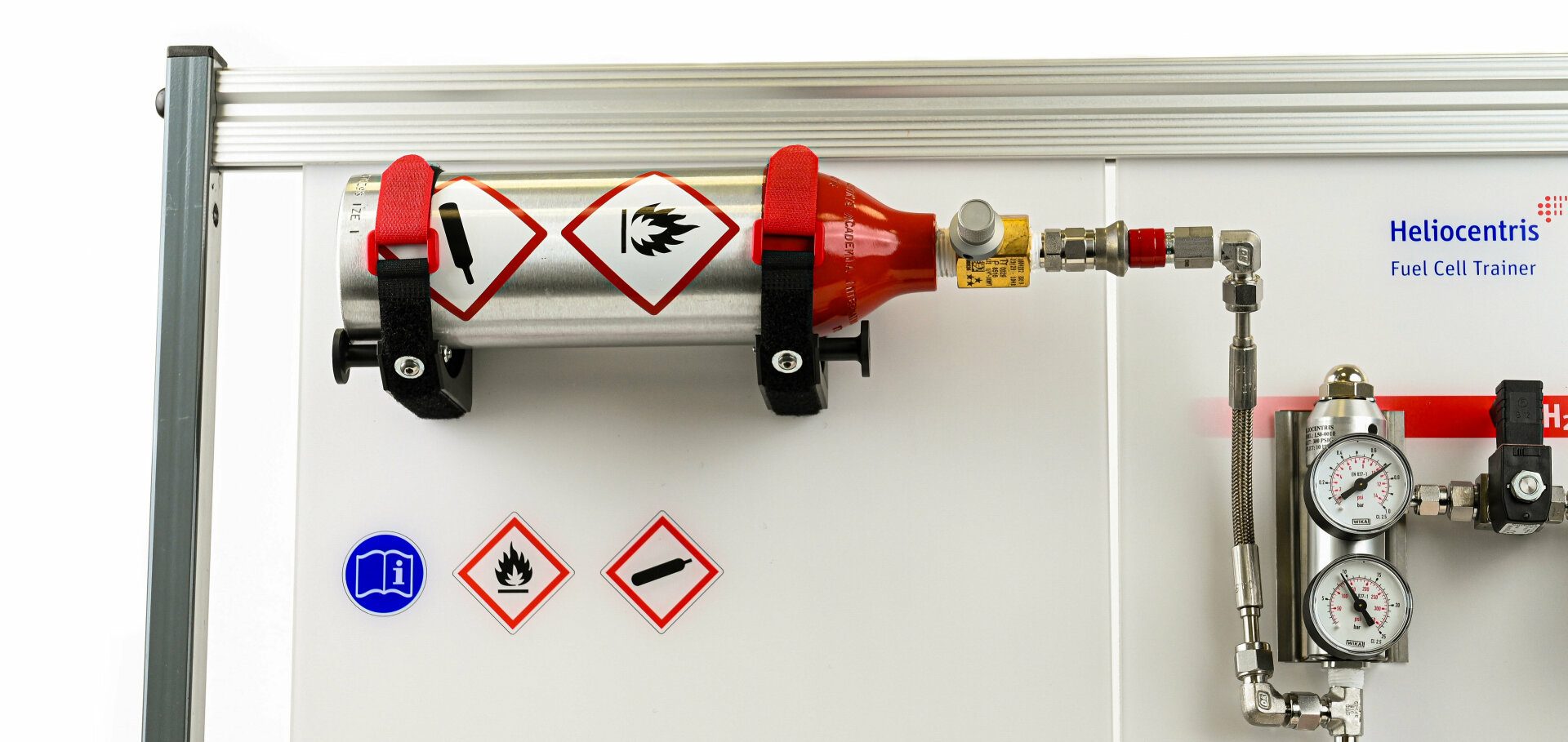
Introduction
In recent years, hydrogen has come to the forefront as a leading energy vector for a decarbonized and sustainable energy future. Suppose hydrogen is to become a mainstream energy vector. In that case, we need to find energy and cost-efficient methods to store the hydrogen produced from water electrolyzers and other sources and supply it to fuel cells to generate electricity and heat on demand. Hydrogen can be stored and supplied as a compressed gas, in liquefied form, or using metal hydrides. In this article, we will take a deep dive into the fundamentals of metal hydride storage and their current technological status. By the end, you will be able to contrast metal hydrides with the other forms of hydrogen storage.
Hydrogen is a gas under ambient conditions with a very low density of 0.082 kg/m3 at 25 oC and 1 atm). To store it in the gaseous form, hydrogen needs to be compressed to high pressure (350-750 bar) and filled into high-pressure tanks. For hydrogen storage in the liquid form, the gas needs to be cooled to -252.8 oC and must be stored cryogenically at this temperature. Both these processes, i.e., compressing the gas to high pressure and liquefying the gas, require high energy expenditure. For example, estimates indicate that compressing hydrogen to 750 bar might require 18 MJ/kg (nearly 15% of hydrogen’s lower heating value). [1] Additionally, the handling and transportation of high-pressure hydrogen tanks also present major safety concerns due to their highly explosive nature. For liquid hydrogen storage, the energy required for liquefaction is nearly 20-50% of the lower heating value of hydrogen. [1] Also, hydrogen boil-off losses due to heat transfer from the surroundings are inevitable. Both compressed gas and liquid storage have relatively low volumetric energy density (0.8 - 2.2 kWh/L). This presents a major challenge, especially for automotive applications, where stringent targets on the volume and fuel capacity need to be met.
What is Metal Hydride Storage?
Some metals can form hydrides with hydrogen and can be used to store hydrogen. These materials store hydrogen in a dissolved atomic state between the solid lattice and can reversibly absorb and release hydrogen. The general reaction for the metal hydride process can be written as, (1)
\[M + (x/2) H_2 ⇐⇒ MHx\]Metal hydrides can be classified according to their composition as elemental, intermetallic, or complex. Elemental metal hydrides comprise of a single metal which bonds with hydrogen. Most common elemental hydrides include MgH2, AlH3, and TiH2. Elemental metal hydrides (except TiH2) are cheap and abundant with a high theoretical storage capacity (up to 10.1 wt%) making them an attractive option for hydrogen storage. However, the high operating temperature and pressure required for absorption and desorption and high specific enthalpy make them unsuitable for mobile applications such as automotive vehicles. TiH2 on the other hand has a low storage capacity of only around 1 wt%.
Intermetallic hydrides consist of two or more metals or metal alloys forming bonds with hydrogen. The two metals are typically chosen such that one forms a strong hydride and the other forms a weak hydride and the composition is varied to achieve good thermodynamic properties for the absorption and desorption of hydrogen. Most common intermetallic hydrides (having a general form AxByHz) have the form AB, AB2 and AB5 where A and B are the two metals forming the hydrides. Some commonly used intermetallic hydrides are TiFe, TiMn2 and LaNi5. These materials demonstrate good cycling stability and can be operated at relatively low temperatures and pressures. These materials are, therefore, suitable for low-grade heat sources (heat rejected at low temperatures). The main disadvantage of these materials is their low storage capacity (< 2 wt%) resulting in a significant increase in the weight of the storage tanks. Although LaNi5 is the most suitable choice in terms of operating conditions, stability, and hydrogenation rate, the use of rare earth metal (La) makes it infeasible for large-scale hydrogen storage.
Complex hydrides are made of a light metal (such as Na, Li, etc.) and a complex anion consisting of hydrogen. Some common anions with hydrogen include [AlH4]-, [BH4]- and [NH2]-. Complex metal hydrides have a high storage capacity (LiBH4 has a storage capacity of 18.5 wt%) which exceeds the US Department of Energy target for specific energy of onboard hydrogen storage in light-duty vehicles. The main challenges with complex metal hydrides are slow hydrogenation kinetics (discussed in the next section) and poor reversibility (desorbing hydrogen). These materials are also flammable and spontaneously ignite upon exposure to oxygen making them difficult to handle.
Metal hydrides have significantly higher (4-5 times) volumetric energy density compared to compressed gas and liquid hydrogen storage making them an attractive solution for hydrogen storage. The key challenges with metal hydrides are their absorption and desorption kinetics, operating temperature (and pressure), and stability and resistance to impurities. [2]
How do Metal Hydrides Store and Release Hydrogen?
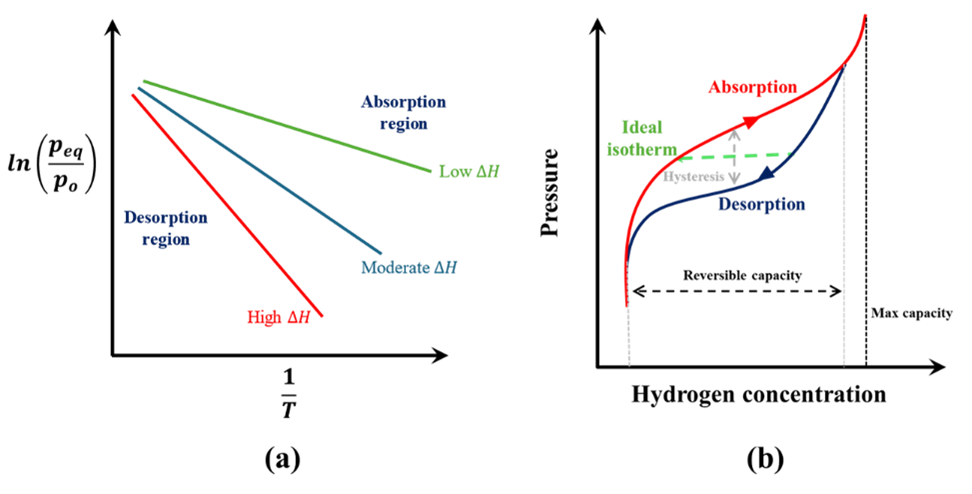
In general, metal hydrides absorb hydrogen at high gas pressure while releasing heat. To supply hydrogen from the metal hydrides, heat must be supplied to desorb the hydrogen. To understand the operating temperature and pressure ranges for different metal hydrides, we first need to understand the Van’t Hoff plot and the pressure-composition isotherm. Fig 1a shows a typical Van’t Hoff plot which describes the relation between the equilibrium pressure of absorption and desorption of hydrogen in metal hydrides as a function of temperature. The relationship between the equilibrium pressure (peq) and temperature (T ) can be described using, where po is a reference pressure, ∆H is the enthalpy of the reaction, ∆S is the entropy of reaction and R is the universal gas constant. The entropy of reaction primarily depends on the entropy change of hydrogen and therefore, it is nearly the same for all metal hydrides. [2] The equilibrium line divides the absorption (on the right) and desorption (on the left) regions. For hydrogen to absorb into the metal, the gas pressure needs to be higher than the equilibrium point. Similarly, for the desorption of hydrogen, the gas pressure needs to be lower than the equilibrium pressure.
\[ln \left({p_{eq}\over p_o}\right) = -{∆H \over RT} + {∆S \over R}\]As can be seen from Eq. (2), the slope of the equilibrium line in the Van’t Hoff plot depends on the enthalpy of the reaction. The reaction enthalpy is a critical parameter defining the operating temperature and pressure for the metal hydrides. For elemental (such as MgH2) and complex (such as LiBH4) metal hydrides, the enthalpy is high (resulting in higher operating temperatures) while for intermetallic hydrides the enthalpy is typically lower.
The enthalpy is also a key parameter when it comes to the design of the thermal management system for the metal hydride storage canister. Absorption of hydrogen into the metal is an exothermic reaction and therefore, temperatures below the equilibrium temperature favor absorption. For desorption of hydrogen, heat must be supplied to the metal hydride to release hydrogen at a desired pressure and therefore, temperatures above the equilibrium temperature at a given pressure favor desorption. The reaction enthalpy is a measure of the amount of heat released and required during the absorption and desorption process respectively. Therefore, high reaction enthalpy values of elemental metal hydrides mean that a better thermal management system must be designed when using these materials to remove and add heat at a high temperature. Without an efficient thermal management system, the heat produced during absorption can result in a temperature increase in the canister, thereby inhibiting the absorption reaction. Conversely, during desorption, insufficient heat supply can result in lower desorption rates and a drop in the hydrogen supply pressure. Lower reaction enthalpies, resulting in ambient operating temperature, for intermetallic hydrides is a key reason for them being a popular choice for commercial hydrogen storage canisters.
Fig. 1b shows a sketch of a typical pressure-composition isotherm. The maximum capacity denotes the hydrogen composition when the metal has fully been converted to a metal hydride. The reversible capacity denotes the ideal working range of the metal hydrides where the metal hydride can be reversibly cycled to store (absorb) and supply (desorb) hydrogen. Ideally, the absorption-desorption processes should occur at a constant pressure. However, a real isotherm differs in a few aspects. First, the absorption and desorption paths show hysteresis, i.e., hydrogen supply during desorption is at a lower pressure than the absorption phase. Second, the absorption and desorption paths have a finite slope (instead of being parallel to the x-axis). The slope of the absorption/desorption path depends on the type of materials and can be controlled by doping the metal hydrides with additional materials. For example, doping TiMn2 with copper resulted in a decrease in the slope of the absorption/desorption path. [3]
The reaction kinetics of the absorption and desorption reaction also play a crucial role in determining the feasibility of metal hydrides for hydrogen storage. The reaction kinetics directly impact the refueling and extraction times and therefore, fast kinetics are desired to ensure low refueling and extraction time. Metal hydrides have slow kinetics resulting in much longer refueling and extraction time compared to compressed gas or liquid hydrogen storage systems. Consequently, significant research efforts are directed towards doping metal hydrides with transition elements (such as Mn, Co, V, Cu, Fe) to improve the reaction kinetics. [2] The reaction kinetics can also be increased by improving the heat transfer to and from the metal hydride. [4]
Conclusion
Metal hydrides provide an energy-efficient and safe method to store hydrogen (because hydrogen is bound to a metal or alloy). Metal hydrides have much higher volumetric energy density compared to compressed gas or liquid hydrogen storage making them a viable option to meet targets for automotive applications. However, metal hydrides have low gravimetric storage capacity (compared to liquid or gaseous hydrogen storage) which results in a significant mass of the metal or alloy being added to the system. For stationary applications, low-cost materials, such as MgH2, could be used in conjunction with a well-designed thermal management system. For portable applications, research is focusing on the development of metal hydrides to improve their reaction kinetics, operating temperature range, stability, and tolerance to impurities.
Mayank Sabharwal
Assistant Professor at the University of Calgary
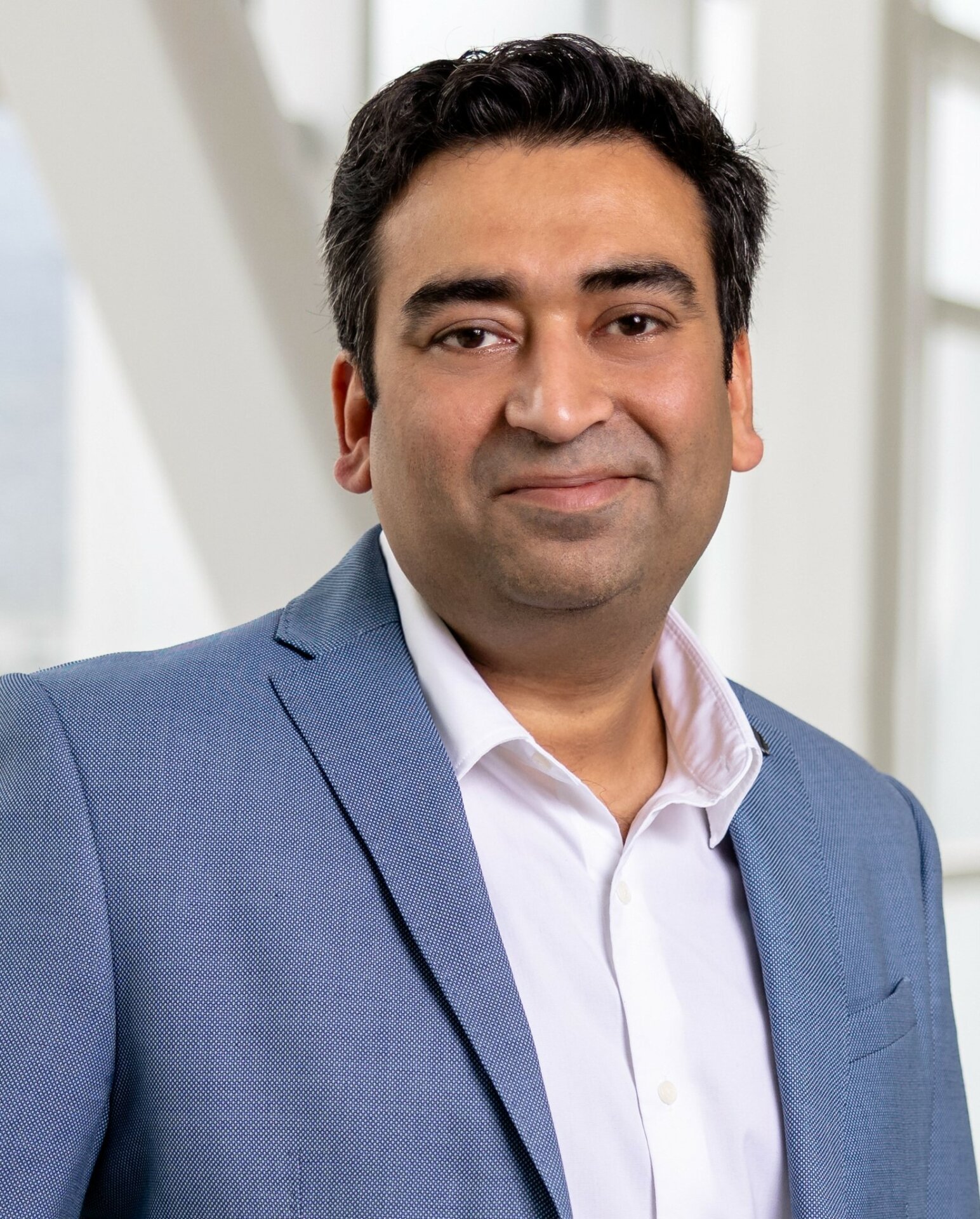
Mayank Sabharwal is an Assistant Professor and Chair of Sustainable Hydrogen in the Department of Chemical and Petroleum Engineering at the University of Calgary. He received his PhD from the Mechanical Engineering Department at the University of Alberta in 2019. After his PhD, he was a postdoctoral fellow at Paul Scherrer Institute in Switzerland (from 2019 to 2021) and Lawrence Berkeley National Laboratory in USA (from 2021 to 2023). His current research focuses on material innovation and novel electrode architecture development for different electrochemical systems related to energy storage and hydrogen production and utilization. His research group develops operando imaging tools and multiscale numerical models to understand the transport processes in these systems.
References
[1] Chris Drawer, Jelto Lange, and Martin Kaltschmitt. Metal hydrides for hydrogen storage–identification and evaluation of stationary and transportation applications. Journal of energy storage, 77:109988, 2024.
[2] Nejc Klopcic, Ilena Grimmer, Franz Winkler, Markus Sartory, and Alexander Trattner. A review on metal hydride materials for hydrogen storage. Journal of Energy Storage, 72:108456, 2023.
[3] Bin-Hong Liu, Dong-Myung Kim, Ki-Young Lee, and Jai-Young Lee. Hydrogen storage properties of timn2-based alloys. Journal of alloys and compounds, 240(1-2):214–218, 1996.
[4] Huy Quoc Nguyen and Bahman Shabani. Review of metal hydride hydrogen storage thermal management for use in the fuel cell systems. International Journal of Hydrogen Energy, 46(62):31699–31726, 2021.